The brain’s capacity to reorganize and adapt after damage is known as neuroplasticity or brain plasticity.
Take-home Messages
- Brain plasticity, also known as neuroplasticity, is the brain’s biological, chemical, and physical capacity to reorganize its structure and function.
- Neuroplasticity occurs due to learning, experience, and memory formation or due to damage to the brain.
- Learning and new experiences cause new neural pathways to strengthen, whereas neural pathways used infrequently become weak and eventually die. This process is called synaptic pruning.
- Although traditionally associated with changes in childhood, recent research indicates that mature brains continue to show plasticity due to learning.
- Neuroplasticity provides protective effects in managing traumas during human development (Cioni et al., 2011). Also, learning music or second languages can increase neuroplasticity (Herholtz & Zatorre, 2012).
- Plasticity allows the brain to cope better with the indirect effects of brain damage resulting from
inadequate blood supply following a stroke. - Fundamentally, the nervous system needs to rearrange itself to adapt to its unfolding situation. The genes program the body to have neuroplasticity so that animals can survive in unpredictable environments.
Neuroplasticity, also called brain plasticity, refers to the capacity of the brain to change and adapt in structure and function in response to learning and experience.
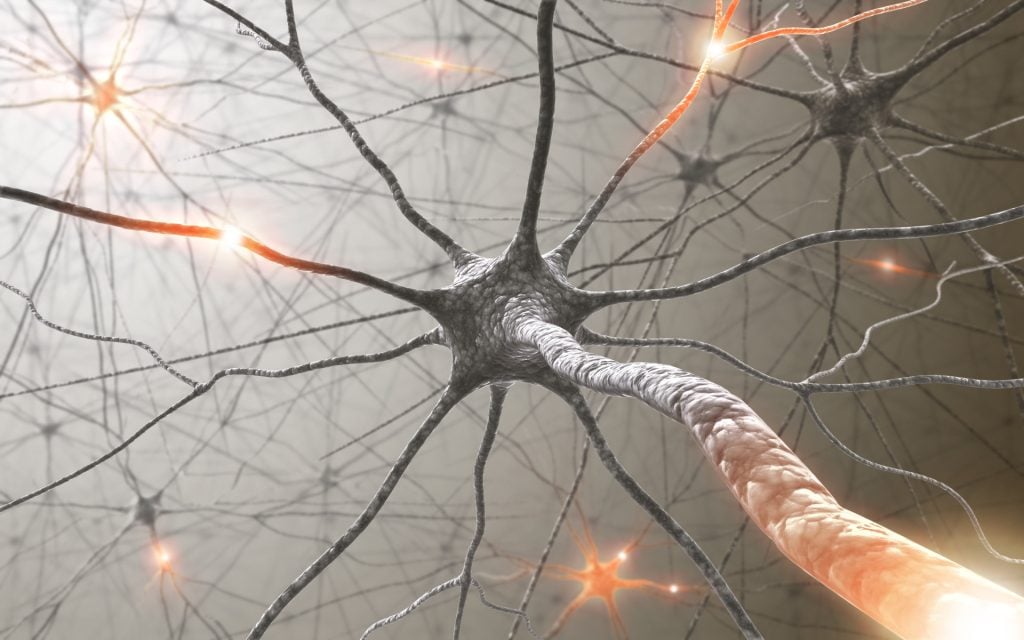
The brain possesses a remarkable ability to rewire itself. These changes range from individual neuron pathways making new connections to systematic adjustments like cortical remapping.
This occurs in all healthy people, especially children, after various problems like brain injuries.
Early Theories
Early experimental work on neuroplasticity was conducted by an eighteenth-century Italian scientist, Michele Malacarne, who discovered that animals made to learn tasks would develop larger brain structures (Rosenzweig, 1996).
The first theoretical notions of neural plasticity were developed in the nineteenth century by William James, a psychology pioneer. James wrote about this topic in his 1890 book The Principles of Psychology (James, 1890).
In the twentieth century, influential neuroscientist Santiago Ramón y Cajal proposed that neurons in adults break down and rebuild (Fuchs & Flügge, 2014).
Modern Theories
Progress of the idea – modern theories: Modern experimental instruments like imaging tools have yielded enough information to develop improved theories.
Scientists now think that neuroplasticity occurs throughout all life stages, with extensive capacities from childhood development to healing diseases (Doidge, 2007).
The brain can rearrange itself in terms of the functions it carries out and the basic underlying structure (Zilles, 1992).
Functional Plasticity
Functional Recovery After Brain Trauma
Functional plasticity is the brain’s ability to move functions from a damaged area of the brain after trauma, to other undamaged areas. Existing neural pathways that are inactive or used for other purposes take over and carry out functions lost because of the injury.
After a brain injury, such as an accident or stroke, the unaffected brain areas can adapt and take over the functions of the affected parts. This process varies in speed, but it can be fast in the first few weeks (phase of spontaneous recovery) then it becomes slower.
It can be helped by rehabilitation, and the nature of rehabilitation programs varies with the type of injury, from retraining some types of movement to speech therapy.
There are ways through which brain plasticity can enable brain-damaged people to regain some of their past capacities. Each of the approaches through which the nervous system adapts its functionality has differences in how it occurs and in which patients it occurs.
Axonal sprouting
Functional plasticity can occur through a process termed axonal sprouting, where undamaged axons grow new nerve endings to reconnect the neurons, whose links were severed through damage.
Undamaged axons can also sprout nerve endings and connect with other undamaged nerve cells, thus making new links and new neural pathways to accomplish what was a damaged function.
Homologous Area Adaptation
Although each brain hemisphere has its own functions, if one brain hemisphere is damaged, the intact hemisphere can sometimes take over some of the functions of the damaged one.
In homologous area adaptation, brain-behavior becomes active in the equivalent part on the opposite side of the brain from where it usually occurs (Grafman, 2000). If it normally occurs on the right side, it would move to the left side, and vice versa.
This functional neuroplasticity occurs more often in children than in adults. Shifting over a module to the opposite side displaces some of the functionality that was originally there.
As a result, the two functions may become less effective, contaminating each other.
Cross-Modal Reassignment
Cross-modal reassignment occurs when the brain uses an area that would normally process a certain type of sensory information (such as sight) for a different type of sensory information instead (such as sound).
When a brain region does not receive sensory data as expected, say because a person has become blind, this brain region may become repurposed for another sense, like touch.
This can enable blind people to “see” Braille text with their fingers (Grafman, 2000).
Also, some blind people learn to reuse their visual centers for hearing sounds, thus becoming capable of “echolocation” to navigate around environments (Thaler & Goodale, 2010).
Map Expansion
In map expansion, the brain notices that a certain area gets extensive use, so it expands this area (Grafman, 2000). This is comparable to how the body can notice that certain muscles get more use (such as those involved in an often-played sport), then grows those muscles larger.
When a person often engages in an activity or experience, this produces enlargement of the associated brain region. Brain growth occurs right away, so neuroscientists can detect it through brain imaging technologies while it occurs (Grafman, 2000).
Compensatory Masquerade
Compensatory masquerade involves the brain reusing a component to conduct a mental operation other than what it would typically do.
For example, suppose a person suffers a brain injury with some functionality lost. In that case, this person may be able to reuse a different method behind the scenes, like finding one’s way by remembering directions instead of by sense of location (Grafman, 2000).
Evidence For Functional Plasticity
Homologous Area Adaptation:
Case studies of stroke victims who have experienced brain damage and thus lost some brain functions have shown that the brain has the ability to re-wire itself with undamaged brain sites taking over the functions of damaged brain sites.
Thus, neurons next to damaged brain sites can take over at least some of the functions that have been lost.
A youth with a right parietal lobe injury wound up with the left parietal lobe taking over some functions normally occurring on the right side. The youth then had difficulty with tasks normally occurring on the left side because some right-side equivalents had taken over left-side brain resources (Grafman, 2000).
Neuronal Unmasking:
Wall (1977) noticed the brain contained ‘dormant synapses’ – neural connections which have no function.
However, when brain damage occurs, these synapses can become activated and open up connections to regions of the brain that are not normally active and take over the neural function that has been lost due to damage.
Structural Plasticity
How Experience Changes Brain Plasticity
Structural neuroplasticity is the brain’s ability to change its physical structure as a result of learning, involving reshaping individual neurons (nerve cells).
During infancy, the brain experiences rapid growth in the number of synaptic connections.
As each neuron matures, it sends out multiple branches; this increases the number of synaptic contacts from neuron to neuron. At birth, each neuron in the cerebral cortex has approximately 2,500 synapses.
By the time a child is three years old, the number of synapses is approximately 15,000 (Gopnick et al. 1999).
As we mature, the connections we do not use are deleted, and the ones we use frequently are strengthened, called neural pruning (Purcell & Zukerman, 2011). This process continues throughout our life.
While plasticity occurs throughout life, it is especially relevant during the early “critical years,” when brain plasticity enables the senses, language, and other skills to develop.
Developmental plasticity
Part of the development of the vision system is genetically hardwired. However, another part of this development depends on neuroplasticity. As a child grows, the incoming information from light sources, such as light reflected off the faces of caregivers, provides necessary cues for the brain to adjust its growth patterns.
The equivalent plasticity-based growth also occurs with the other senses, calibrating the young person to local conditions.
The development of language reveals even more about neuroplasticity. Again, part of this functionality is genetically hardwired, but part depends on environmental feedback. An individual has certain nerve cells programmed to become grammar modules.
For these to function correctly, they require the input of specific grammatical rules from a culture, such as the rules of English or Spanish. Thus, neuroplasticity enables the brain to process language.
How Does Neuroplasticity Work?
At the most basic level, it starts with the production of a new nerve cell (neurogenesis). Then, individual neurons develop new connections with each other.
A neuron works by sending or receiving electrochemical signals from other neurons in the brain.
The way that individual neurons connect to each other controls how the signals get sent, like the routing of messages over the internet or of instructions codes in a computer processor.
As each neuron develops connections to others, this results in growing clusters of cells. The neurons can adjust the level or strength of the signal with connecting neurons.
This ongoing process provides fine-tuning of the neural architecture. Neuroplasticity uses cascades of electrochemical signals that unfold as a result of the expression of genetic codes through cell signal molecules (Flavell & Greenberg, 2008).
Rewiring larger regions, reorganizing the nervous system at multiple levels
Neurons work together at several different levels. Not only individual cells but even clumps within brain regions can grow in greater or lower density.
As cells grow or die in different regions, the relative densities vary. Such variations can provide an even broader adjustment or neuroplasticity in the brain than individual nerve cell connections.
When nerve bundles become broken, through injury or surgery, the brain can regrow these elements (Doidge, 2007). Surprisingly, the brain can reconnect itself in an efficient manner even to deal with sizable upsets. It operates like a plant, able to regrow around lost parts.
The brain can regrow after injury, starting instantly at the molecular level in the injured area (Wall & Wang, 2002). Gradually, the repairs extend through subcortical layers, reaching larger-scale cortical levels of the brain.
This growth occurs throughout the nervous system, including the spine and distributed branches, not only in the brain.
Recurring synaptic connections grow more efficient (cell assembly theory)
Nerve cells work by producing electrochemical activity in the “synapses,” which are gaps connecting the cells together.
As a synaptic connection fires more often, it grows more efficient in what is known as “cell assembly theory.” A phrase describing this phenomenon is “cells that fire together wire together” (Lowel, 1992).
The nerve connections grow stronger when one cell fires before the other rather than when they both fire simultaneously. Sequential firing produces a causal relationship, enabling the nervous system to learn.
As a comparison, internet search engines track which sites link directly to which other sites. The combined directional links of billions of sites produce an efficient map of the internet.
The combined directional links of billions of neurons produce an efficient map of the body and its environment.
Evidence for Structural Plasticity
The famous study by Maguire et al., 2000 demonstrates brain plasticity. She studied 16 London taxi drivers and found an increase in the volume of grey matter in the posterior hippocampus compared to a control group. This area of the brain is involved in short-term memory and spatial navigation.
Further support comes from Mechelli et al. (2004), who found that learning a second language increases the density of grey matter in the left inferior parietal cortex and that the degree of structural reorganization in this area is influenced by the fluency attained and the age at which the second language was learned.
With age, neuroplasticity decreases; however, Mahncke et al. (2006) used a computer training program with older adults with memory impairment and found a significant improvement compared to the control group.
This has potential benefits for society as a brain-plasticity-based intervention targeting normal age-related cognitive decline may delay the time when these people need support in their everyday life.
Learning and new experiences cause new neural pathways to strengthen, whereas neural pathways which are used infrequently become weak and eventually die. Thus brains adapt to changing environments and experiences. Boyke (2008) found that even at 60+, learning a new skill (juggling) resulted in increased neural growth in the visual cortex.
Kuhn (2014) found that playing video games for 30+ minutes per day resulted in increased brain matter in the cortex, hippocampus, and cerebellum. Thus, the complex cognitive demands of mastering video games caused the formation of new synaptic connections in brain sites that control spatial navigation, planning, decision-making, etc.
Davidson (2004) matched eight experienced practitioners of Tibetan Buddhist meditation against 10 participants with no meditation experience. Levels of gamma brain waves were far higher in the experienced meditation group both before and during meditation. Gamma waves are associated with the coordination of neural activity in the brain.
This implies that meditation can increase brain plasticity and cause permanent and positive changes to the brain.
Kempermann (1998) found that rats housed in more complex environments showed an increase in neurons compared to a control group living in simple cages. Changes were particularly clear in the hippocampus – associated with memory and spatial navigation.
A similar phenomenon was shown in a study of London taxi drivers. MRI scans revealed that the posterior portion of the hippocampus was significantly larger than the control group, and the size of the difference was positively correlated with the amount of time spent as a taxi driver (i.e., greater demands on memory = more neurons in this portion of the hippocampus).
Critical Evaluation
Neuroplasticity can explain a broad range of facts about the structure and function of the brain. This notion does, however, have some constraints.
These involve the gradual decline of neuroplasticity with age and certain restrictions regarding how much neural plasticity is possible, even in young, healthy people. Also, scientists have yet to learn many critical aspects of neuroplasticity.
The limits of brain plasticity (decline with age, biological constraints)
Neuroplasticity can only go so far. Non-human animals show many areas of brain plasticity. However, their brains cannot reshape themselves enough to learn a human language or perform advanced mathematics.
Neuroplasticity works on biologically available material, which imposes limitations like only adjusting the specific neural substrate of a cognitive function or adapting a brain function somewhat for a season.
In people whose brains reuse large regions for different operations, such as blind people whose vision centers become useful for touch or sound, this capacity can only work for specific types of processing.
Even people blind from birth would not be able to reuse their color-detection brain cells for touch because, unlike geometry-detection brain cells, these have hard coding for visual input (Grafman, 2000).
Even in healthy individuals, neuroplasticity declines with age (Lu et al., 2004). Over the years, as the body becomes less flexible, so does the brain.
Much neuroplasticity is geared towards enabling younger people to develop an understanding and capacity to act within their surroundings. This stabilizes to some extent in adulthood, even declining in the elderly.
One can see the decline of neuroplasticity in how older people become more fixed in their ways while younger people learn rapidly.
What don’t we know about brain plasticity?
Neuroplasticity has grown over recent centuries into a topic of considerable interest to scientists but remains poorly understood. The brain imaging tools for conducting studies on this topic are still young. As such, much knowledge has yet to be found.
Scientists remain unsure about many of the mechanisms underlying brain plasticity (Grafman, 2000). While a few of the processes have been studied in molecular detail, others have not, and the conceptual understanding of how they take place is a source of ignorance.
Therefore, the technical underpinnings of neuroplasticity represent an area of active interest in which further investigations are being carried out.
Neuroplasticity, the freedom of the brain to readjust its routing to enable more effective calculation in response to injury or normal challenges, has shown itself to occur across a range of animals.
However, we still only know some of the brain regions in which it occurs, some of the mechanisms through which it happens, and some of the costs and advantages that it offers.
Animal adaptations
Animals other than humans exhibit neuroplasticity as much as humans do, or in some cases, even more.
Animals evolved to survive over periods of years through recurring cycles of weather and other environmental conditions. As such, some species exhibit neuroplasticity in cyclical patterns (Nottebohm, 1981).
Brain regions that navigate the environment, like the hippocampus, often grow during mating season (Nottebohm, 1981). Also, some birds’ brain centers for singing mating songs grow during this period.
Other animals’ brains develop differently depending on the season, such as adjusting when to lay more eggs or become pregnant (Wayne et al., 1998).
Behavioral or environmental modification
Several alterations to one’s behaviors or environmental conditions can affect the brain’s structure. These include sports or other physical activities, meditation, using drugs, or pollution.
When a person becomes physically active, this affects the brain and the rest of the body. Aerobic activities like running or bicycling increase the brain’s rate of producing neurons (Gomez-Pinilla & Hillman, 2013). This results in better awareness of one’s surroundings, decision-making, affect, and other mental functions.
Meditation practices, which involve focusing attention, can also invoke neuroplasticity (Lutz et al., 2004). In this case, the brain increases its capacity for controlling emotions or awareness.
Drugs, including alcohol, illicit drugs, and certain medications, can affect the brain (Ganguly & Poo, 2013). These substances often produce chemical adjustments in the brain, which can last well beyond the duration of drug use, even for decades.
In some cases, like heavy drinking, entire brain regions can restructure themselves to recover lost functionality.
The brain responds to the environment as the main part of its normal function. As such, the brain can also undergo harm from environmental problems.
Air pollution, heavy metals, and other pollutants can inhibit brain development. For example, air pollution can destroy brain cells, reducing cognitive function (Calderón-Garcidueñas, 2002).
References
Bruel-Jungerman, E., Davis, S., & Laroche, S. (2007). Brain Plasticity Mechanisms and Memory: A Party of Four. The Neuroscientist, 13 (5), 492–505. https://doi.org/10.1177/1073858407302725
Calderón-Garcidueñas, L., Azzarelli, B., Acuna, H., Garcia, R., Gambling, T. M., Osnaya, N., Monroy, S., Del Rosario Tizapantzi, M., Carson, J. L., Villarreal-Calderon, A., & Rewcastle, B. (2002). Air Pollution and Brain Damage. Toxicologic Pathology, 30 (3), 373–389. https://doi.org/10.1080/01926230252929954
Cioni, G., D’Acunto, G., & Guzzetta, A. (2011). Perinatal brain damage in children. Progress in Brain Research, 139–154. https://doi.org/10.1016/b978-0-444-53884-0.00022-1
Craik, F. I. M., & Bialystok, E. (2006). Cognition through the lifespan: mechanisms of change. Trends in Cognitive Sciences, 10 (3), 131–138. https://doi.org/10.1016/j.tics.2006.01.007
Doidge, N. (2007). The Brain That Changes Itself: Stories of Personal Triumph from the Frontiers of Brain Science (Reprint ed.). Penguin Books.
Flavell, S. W., & Greenberg, M. E. (2008). Signaling Mechanisms Linking Neuronal Activity to Gene Expression and Plasticity of the Nervous System. Annual Review of Neuroscience, 31 (1), 563–590. https://doi.org/10.1146/annurev.neuro.31.060407.125631
Freed, W., de Medinaceli, L., & Wyatt, R. (1985). Promoting functional plasticity in the damaged nervous system. Science, 227 (4694), 1544–1552. https://doi.org/10.1126/science.3975624
Fuchs, E., & Flügge, G. (2014). Adult Neuroplasticity: More Than 40 Years of Research. Neural Plasticity, 2014, 1–10. https://doi.org/10.1155/2014/541870
Ganguly, K., & Poo, M.-. (2013). Activity-Dependent Neural Plasticity from Bench to Bedside. Neuron, 80 (3), 729–741. https://doi.org/10.1016/j.neuron.2013.10.028
Grafman, J. (2000). Conceptualizing functional neuroplasticity. Journal of Communication Disorders, 33 (4), 345–356. https://doi.org/10.1016/s0021-9924(00)00030-7
Herholz, S. C., & Zatorre, R. J. (2012). Musical Training as a Framework for Brain Plasticity: Behavior, Function, and Structure. Neuron, 76 (3), 486–502. https://doi.org/10.1016/j.neuron.2012.10.011
James, W. (1890). The Principles of Psychology, Vols. 1-2.
Lowel, S. (1992, January 10). Selection of intrinsic horizontal connections in the visual cortex by correlated neuronal activity. Science. https://science.sciencemag.org/content/255/5041/209.long
Lu, T., Pan, Y., Kao, S.-Y., Li, C., Kohane, I., Chan, J., & Yankner, B. A. (2004). Gene regulation and DNA damage in the ageing human brain. Nature, 429 (6994), 883–891. https://doi.org/10.1038/nature02661
Lutz, A., Greischar, L. L., Rawlings, N. B., Ricard, M., & Davidson, R. J. (2004). Long-term meditators self-induce high-amplitude gamma synchrony during mental practice. Proceedings of the National Academy of Sciences, 101 (46), 16369–16373. https://doi.org/10.1073/pnas.0407401101
Nottebohm, F. (1981). A brain for all seasons: cyclical anatomical changes in song control nuclei of the canary brain. Science, 214 (4527), 1368–1370. https://doi.org/10.1126/science.7313697
Purcell, A., & Zukerman, W. (2011, August 17). Brain’s synaptic pruning continues into your 20s. New Scientist. https://www.newscientist.com/article/dn20803-brains-synaptic-pruning-continues-into-your-20s/
Rosenzweig, M. R. (1996). Aspects of the Search for Neural Mechanisms of Memory. Annual Review of Psychology, 47 (1), 1–32. https://doi.org/10.1146/annurev.psych.47.1.1
Thaler, L., Arnott, S. R., & Goodale, M. A. (2010). Human Echolocation I. Journal of Vision, 10 (7), 1050. https://doi.org/10.1167/10.7.1050
Wall, J. T., Xu, J., & Wang, X. (2002). Human brain plasticity: an emerging view of the multiple substrates and mechanisms that cause cortical changes and related sensory dysfunctions after injuries of sensory inputs from the body. Brain Research Reviews, 39 (2–3), 181–215. https://doi.org/10.1016/s0165-0173(02)00192-3
Wayne, N. L., Kim, Y. J., & Yong-Montenegro, R. J. (1998). Seasonal Fluctuations in the Secretory Response of Neuroendocrine Cells of Aplysia californicato Inhibitors of Protein Kinase A and Protein Kinase C. General and Comparative Endocrinology, 109 (3), 356–365. https://doi.org/10.1006/gcen.1997.7040
Zilles, K. (1992). Neuronal plasticity as an adaptive property of the central nervous system. Annals of Anatomy – Anatomischer Anzeiger, 174 (5), 383–391. https://doi.org/10.1016/s0940-9602(11)80255-4